We are the professional home for a global network of engineers and scientists who represent the photonics community.




Awards
Announcements for Recipients and Submission Deadlines for Upcoming Awards
Our awards program has been established to recognize outstanding achievements and for those accomplishments which enhance the quality of life for all people throughout the world.
Purpose &
Connectivity
IEEE Photonics Society is a volunteer-driven organization.
We’re made up of researchers, academics, students, and industry professionals who are dedicated to transforming breakthroughs in photonics to revolutionize our daily lives. From our conferences and publications to networking opportunities, chapters, and awards—this is a community with a purpose and sense of belonging.
Stay Connected
Don’t miss out! Follow us on social or sign up for email and have news and announcements delivered to your inbox.
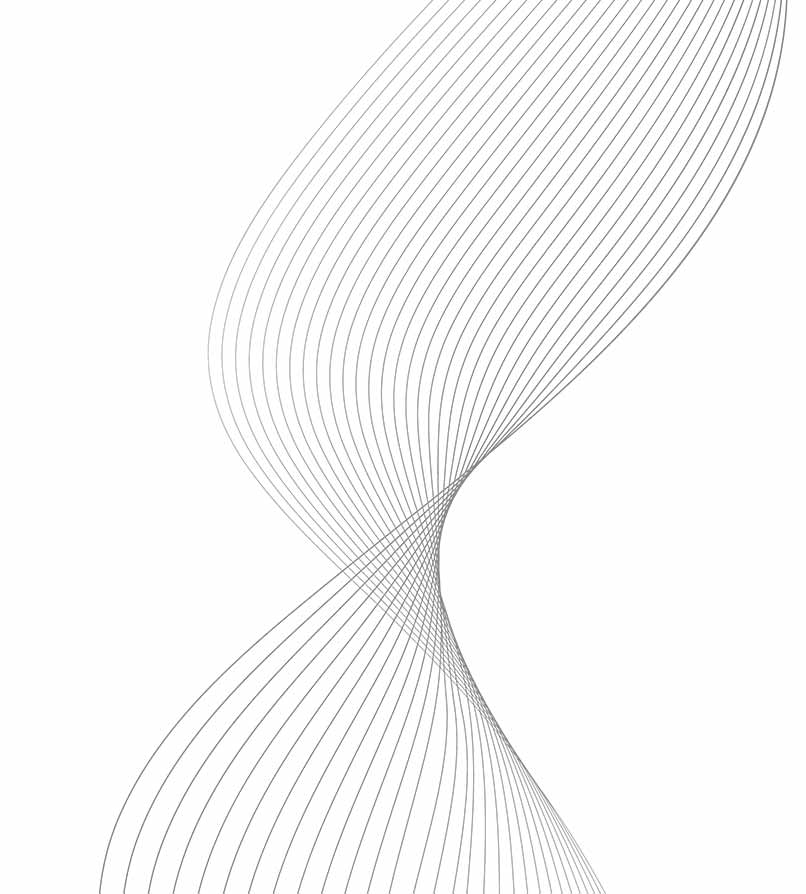